Introduction
Chronotropic incompetence (CI) is a condition characterized by the heart’s inability to adequately increase its rate in response to increased physical activity or metabolic demand. This common issue, particularly prevalent in patients with cardiovascular disease, significantly contributes to exercise intolerance, diminishes quality of life, and serves as an independent predictor of major adverse cardiovascular events and overall mortality. Despite its clinical significance, CI is often underdiagnosed and overlooked in routine practice. This oversight may stem from the varied definitions of CI, the confounding effects of aging and medications, and the necessity for formal exercise testing to confirm diagnosis. This article provides a comprehensive review of CI, encompassing its definition, underlying mechanisms, diagnostic approaches, and current treatment strategies, with a particular focus on its critical role in heart failure (HF). Recognizing CI is crucial as it is a common, objectively diagnosable condition using widely accessible and cost-effective methods. Importantly, CI is potentially treatable, and effective management can lead to substantial improvements in patients’ exercise tolerance and overall quality of life.
The Vital Role of Heart Rate in Exercise Performance
Physical work capacity is a key determinant of an individual’s quality of life [1], largely facilitated by the body’s ability to increase oxygen uptake (VO2) [2]. During maximal aerobic exercise in healthy individuals, VO2 can increase approximately fourfold [2]. This increase is primarily achieved through a 2.2-fold increase in heart rate (HR), along with a smaller 0.3-fold increase in stroke volume, and a 1.5-fold increase in arteriovenous oxygen difference [2]. The substantial contribution of heart rate to enhanced VO2 highlights its paramount importance in sustaining aerobic exercise [3]. Consequently, CI can be a primary or significant contributing factor to severe, symptomatic exercise intolerance in affected individuals.
Understanding Heart Rate Control
Heart rate regulation is a dynamic process, reflecting the delicate balance between the sympathetic and parasympathetic branches of the autonomic nervous system. While the sinoatrial (SA) node’s intrinsic firing rate is approximately 100 beats per minute, resting HR in humans is typically lower (60-80 bpm) due to the dominant influence of the parasympathetic nervous system via the vagus nerve. Elevated resting HR, often resulting from increased sympathetic or decreased parasympathetic tone, has been linked to a higher risk of cardiovascular death, ischemic heart disease, and sudden cardiac death in both men and women, even in asymptomatic populations [4, 5]. Furthermore, a resting HR of 70 bpm or higher is associated with increased mortality in patients with stable coronary artery disease and left ventricular (LV) dysfunction [6, 7].
A robust HR response is essential for precisely matching cardiac output to metabolic demands during physical exertion [4]. An impaired chronotropic response can manifest as failure to reach maximal HR, inadequate submaximal HR, or HR instability during exercise. These conditions are relatively common, especially in patients with sick sinus syndrome, atrioventricular block, coronary artery disease, and heart failure (HF) [4].
Following exercise cessation, a rapid HR decline occurs due to sympathetic withdrawal and increased parasympathetic activity. Delayed HR recovery after exercise is a significant indicator of increased all-cause mortality risk across various populations, including both asymptomatic and diseased individuals [8], even after adjusting for cardiovascular disease severity, LV function, and exercise capacity [9]. While several methods exist for evaluating HR recovery, a widely accepted threshold for increased mortality risk is a decrease in HR of less than 10 beats per minute from peak exercise to 1 minute into passive supine recovery [10].
Conversely, highly trained athletes often exhibit a rapid and pronounced HR drop of ≥30-50 beats during the first minute of recovery from intense exercise [11]. The speed and extent of HR recovery are closely related to parasympathetic tone. This relationship was elegantly demonstrated in a study comparing athletes, healthy subjects, and HF patients. Athletes and healthy subjects showed a biexponential HR recovery pattern, with a sharp initial decline followed by a slower decrease (Figure 1A). However, this initial rapid decline was absent when the same subjects were tested after atropine administration, highlighting the role of parasympathetic activity (Figure 1B) [11].
Figure 1. Parasympathetic Tone and Heart Rate Recovery
Figure 1: Influence of parasympathetic tone on heart rate recovery. A. Heart rate trends during the initial 3 minutes post-exercise across athletes, normal subjects, and heart failure patients. Biexponential recovery is evident in athletes and normal subjects, absent in heart failure patients. B. Post-atropine administration, the initial steep decline diminishes. Adapted from Lauer MS, Cleve Clin J of Med. 2009;76:S18-S22.
The Framingham Offspring study, a long-term study following nearly 3,000 healthy individuals for 15 years, revealed that those in the highest quintile of HR recovery at 1 minute post-exercise had significantly lower risks of coronary heart disease and cardiovascular disease (hazard ratios of 0.54 and 0.61, respectively) compared to those in the lower quintiles [12]. Similarly, the MRFIT study demonstrated that delayed HR recovery (≤12 bpm at 1 minute) was associated with a 55% increase in all-cause mortality [13]. A 23-year follow-up study of asymptomatic working men undergoing exercise stress testing [14] identified resting HR >75 bpm, HR increase from rest to peak exercise <89 bpm, and HR decrease <25 bpm after exercise cessation as independent predictors of fatal myocardial infarction. These findings underscore the strong association between autonomic imbalance, reflected in HR responses at rest, during, and after exercise, and increased risk of adverse cardiovascular outcomes and sudden death [8].
Age and Gender Effects on Heart Rate Response to Exercise
Resting HR remains relatively stable with age in adulthood. However, a notable and predictable age-related decline in maximal heart rate during exercise occurs in healthy humans and other mammals [3, 15, 16]. This age-related reduction in maximal HR is the most significant age-related change in cardiac function in terms of magnitude and consequence [3, 17, 18], primarily contributing to the age-related decline in peak aerobic exercise capacity [3, 18]. Starting in early adulthood, maximal HR decreases by approximately 0.7 bpm per year in sedentary, recreationally active, and endurance-trained adults [19]. While the exact mechanisms are not fully understood, research suggests that intrinsic HR decreases by 5-6 bpm per decade, indicating minimal parasympathetic tone at rest in older adults [15]. Supporting this, the HR increase following atropine administration is less pronounced in older individuals [17]. Age also affects sympathetic influence on HR response, with increased circulating catecholamines but reduced responsiveness [17]. For instance, isoproterenol doses that increase HR by 25 bpm in young men only elicit a 10 bpm increase in older individuals [17].
Vigorous exercise training does not significantly alter the normal age-related decline in maximal HR, suggesting it is not simply due to reduced physical activity with age [15]. Furthermore, it is not attributed to inadequate sympathetic stimulation, as serum norepinephrine and epinephrine levels are often increased at rest in healthy elderly individuals, and catecholamine levels rise even more under stress than in younger counterparts.
The traditional formula for predicting maximal HR (220 – age) was developed primarily from studies on middle-aged men, some with coronary artery disease and on beta-blockers [19, 20]. This equation exhibits considerable inter-subject variability, with a standard deviation of ±11 bpm [21], increasing to ±40 bpm in patients with coronary heart disease on beta-blockers [22]. Consequently, the Tanaka et al. formula (208 – 0.7 × age) is gaining acceptance for estimating age-predicted maximal HR (APMHR), although it may still underestimate APMHR in older adults (Figure 2) [21].
Figure 2. Age and Maximal Heart Rate Relationship
Figure 2: Relationship between age and maximal heart rate in over 5,000 asymptomatic women, with 95% confidence limits. The equation peak heart rate = 206 − 0.88 (age) was proposed based on this data. From Tanaka H, JACC 2001;37:153-156.
Earlier studies suggested gender differences in heart rate trajectory during exercise and recovery, with the traditional equation overestimating maximal HR in younger women and underestimating in older women [19, 21]. However, a meta-analysis indicated that maximal HR is not significantly affected by gender [21]. A large prospective study in over 5,000 asymptomatic women demonstrated that the traditional equation significantly overestimates maximal HR, leading to the proposal of a new equation: maximal HR = 206 – 0.88(age) [19].
Brawner et al. [22] found the 220 – age equation invalid for patients with coronary heart disease (CHD) on beta-blockers and developed the equation [164 – 0.7(age)] for this population.
While these equations improve maximal heart rate estimations compared to the traditional 220-age approach, they still have substantial standard errors (10-22 bpm). Given the inherent variability in maximal heart rate, single-predictor regression equations like age are unlikely to be perfectly accurate. Increasing predictor variables offers minimal improvement and reduces clinical practicality. Therefore, selecting an equation generated from a population closely matching the patient of interest is recommended. The Tanaka et al. [19] equation is suitable for apparently healthy individuals, and the Brawner et al. [22] equation for those with known or suspected cardiovascular disease. Though imperfect, these are superior to the “220-age” equation and more clinically useful.
Defining, Diagnosing, and Measuring Chronotropic Incompetence
A significant hurdle in CI research and clinical management has been the lack of consistent diagnostic criteria. This inconsistency likely contributes to the wide range (9-89%) in reported CI prevalence [23-26]. A study evaluating over 1,500 patients referred for pacemaker implantation found that using five different CI definitions resulted in prevalence rates ranging from 34% to 87% [27]. CI is most commonly diagnosed when HR fails to reach a certain percentage (typically 85%, 80%, or less often 70%) of the age-predicted maximal HR (APMHR, usually based on the 220-age equation) during a graded exercise test [28-30]. CI is also assessed by evaluating the heart rate reserve, the change in HR from rest to peak exercise. Adjusted HR reserve, calculated as the change in HR from rest to peak exercise divided by the difference between resting HR and APMHR, is also frequently used [31]. Most studies define CI as failure to achieve ≥80% of the HR reserve during a graded exercise test.
However, before diagnosing CI, patient effort level and reasons for exercise test termination must be considered. Patients should be encouraged to exercise to true symptom-limited maximal exertion. Subjective ratings of perceived exertion (RPE) can estimate effort level. Respiratory exchange ratio (RER), derived from expired gas analysis at peak exercise, is the most definitive, objective measure of physiologic effort. RER is reliable and readily available with modern, automated, and moderately priced equipment. RER values range from approximately 1.0 at moderate exertion to 1.15-1.20 during intense, exhaustive exercise. An RER >1.05 generally indicates maximal effort.
Wilkoff et al. [32] utilized expired gas analysis to objectively evaluate CI using the metabolic-chronotropic relationship (MCR), also known as the chronotropic index. MCR is calculated as the ratio of HR reserve to metabolic reserve during submaximal exercise. The advantage of MCR is its adjustment for age, fitness, and functional capacity, and its independence from exercise testing mode or protocol. In healthy adults, the percentage of HR reserve achieved during exercise typically equals the percentage of metabolic reserve. This principle allows for assessing whether HR at any point during exercise is consistent with normal chronotropic function using the following formula:
HRstage = [(220 − age − HRrest)] × (METsstage − 1) / (METspeak − 1) + HRrest |
---|

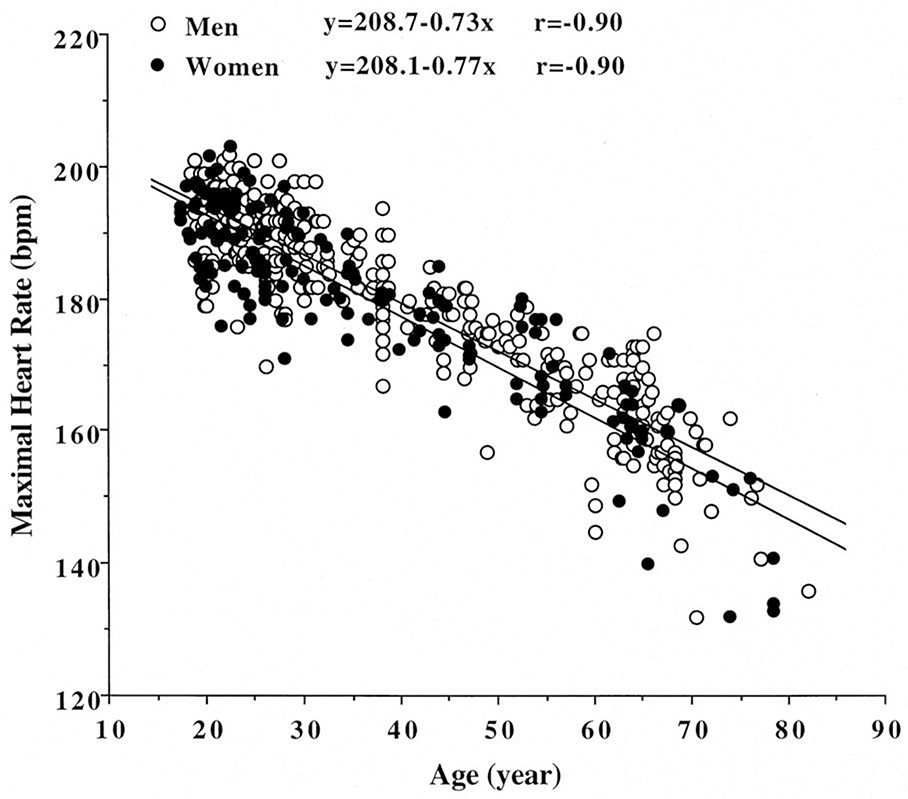
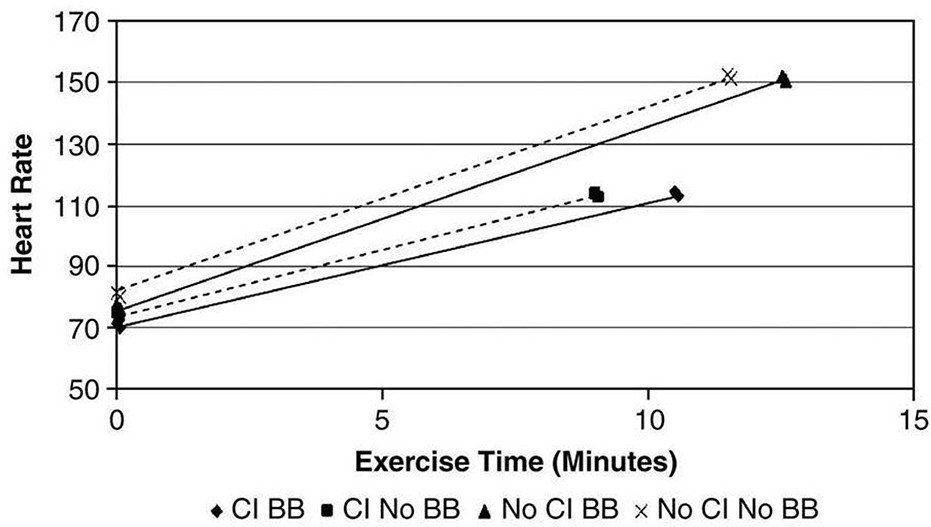
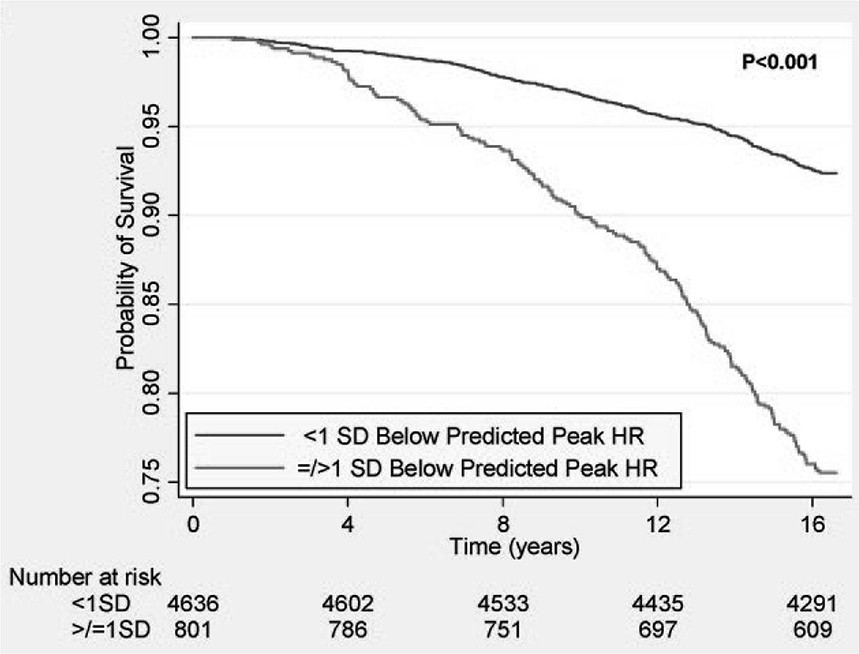
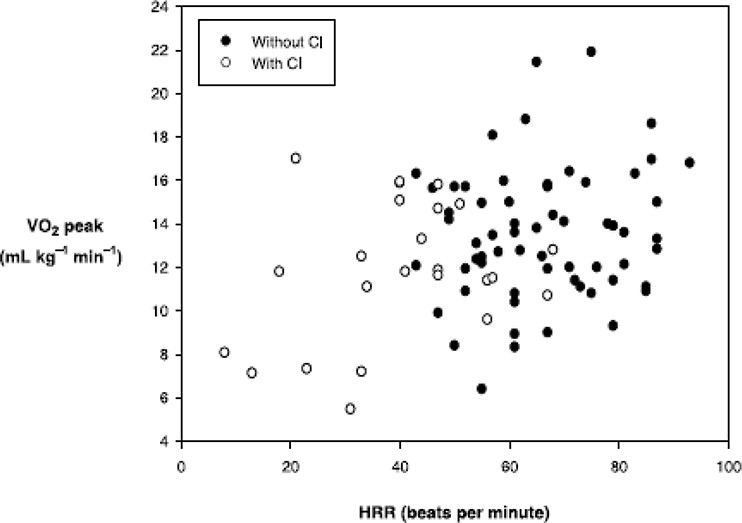
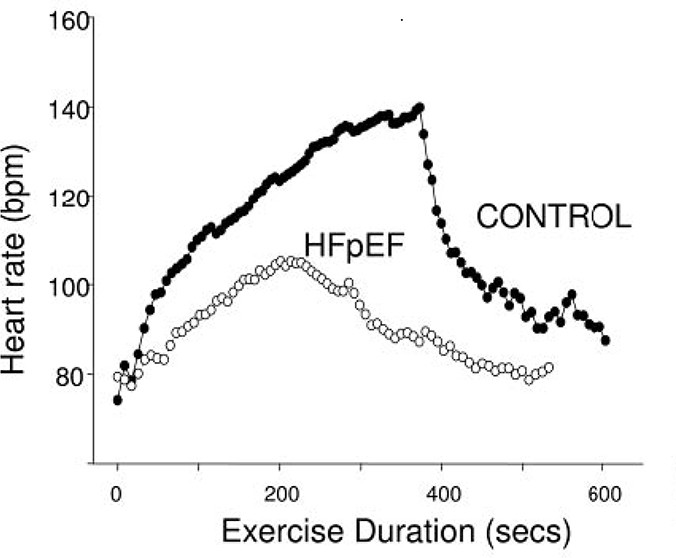
The Wilkoff model predicts a normal MCR slope of 1.0, with a 95% confidence interval between 0.8 and 1.3 [32]. An MCR slope or single-stage MCR value ≤0.80 is indicative of CI.
Therefore, essential data to record during exercise testing for CI evaluation includes: age; resting HR (HRrest); APMHR (220 – age); age-predicted HR reserve (APHRR = APMHR – HRrest); observed maximal HR (HRmax); oxygen consumption (VO2) at each stage and peak effort; and RER. For example, in a 60-year-old subject with peak RER of 0.96 (submaximal effort), data from a submaximal stage (25 watts) with HRrest= 67, HRpeak= 100, HR@25w= 97, MET@25w= 3.3, METpeak= 3.7, yields a CI index of 0.66 (actual HRstage 97/estimated HRstage 147), below the CI cutoff of ≤0.80. The Wilkoff approach [32], combined with other methods, aids CI determination in challenging cases: 1) failure to achieve HRmax ≥ 80-85% of APHRR (or 80-85% HR reserve) despite peak RER > 1.05 (adequate effort); 2) RER < 1.05 (submaximal effort) and MCR relationship of ≤0.80.
While various exercise protocols (Bruce, RAMP, etc.) and modes are suitable, a specific CI exercise protocol used in some labs assesses MCR from two treadmill stages (stage 1 = 1.3 mph & 0.5% grade, stage 2 = 3.0 mph & 1.5% grade). The data collection and analysis described above are then used to evaluate chronotropic responses [32].
Savonen et al. [33, 34] proposed methods to differentiate parasympathetic withdrawal from sympathetic stimulation effects on HR response. This is based on the physiological observation that HR increase below 100 bpm is primarily controlled by parasympathetic withdrawal, while increases above 100 bpm are mainly due to sympathetic activity. Savonen et al. termed this a “delineational” approach. Their research indicates that in men with and without coronary heart disease, HR increase from 40-100% of maximal work capacity predicts mortality and acute myocardial infarction better than peak HR or HR reserve approaches. Similarly, another study [35] showed that blunted HR increase from rest to 33% of maximal work capacity was not as strong a predictor of death as low HR reserve in patients referred for exercise testing. While innovative, these approaches require further validation before widespread clinical application.
Medications and Other Confounding Factors in CI Assessment
Several common cardiovascular medications, including beta-blockers, digitalis, certain calcium channel blockers, and amiodarone, can complicate CI diagnosis [36, 37]. Beta-blockers can induce pharmacological CI, masking underlying intrinsic abnormalities in neural balance [37]. One study [38] suggested a CI threshold of ≤62% of APHRR for HF patients on beta-blockers to reliably identify CI and predict mortality independently. These modified criteria have been used in clinical trials [39]. However, careful assessment of beta-blocker dosage and patient compliance is crucial before applying these modified thresholds.
The need for separate CI criteria for patients on beta-blockers is debated. Some studies have not found beta-blockers, even at high doses, to significantly affect CI occurrence [40]. Figure 3 illustrates the similar relationship between HR reserve and VO2 peak in HF patients, regardless of beta-blocker use. Similarly, Jorde et al. [41] examined the relationship between exercise time and HR during treadmill testing in HF patients. Figure 4 shows that the HR slope is abnormal in HF patients with CI, but beta-blockers do not alter this relationship [42]. Paradoxically, chronic beta-blocker treatment in HF patients may improve chronotropic response by reducing sympathetic tone or increasing beta-receptor activity [43].
Figure 3. Heart Rate Reserve and VO2 peak in HFrEF Patients
Figure 3: Relationship between heart rate change during exercise and VO2peak in patients with HFrEF, showing no significant difference between those taking beta-blockers and those not. From Magri et al; Cardiovascular Therapeutics 2010; In press.
Figure 4. Beta-blocker Impact on Heart Rate and Exercise Time in HF
Figure 4: In HF patients, beta-blockers do not significantly impact the relationship between heart rate and exercise time, regardless of CI presence. From Jorde et al; European J of Heart Failure 2008;96-101.
Chronic atrial fibrillation also complicates CI assessment, and specific diagnostic criteria are lacking. Exercise testing can assess HR response post-pacemaker implantation for CI. Intrinsic HR response can be evaluated in pacemaker patients by temporarily reprogramming or suspending the device with a magnet, ensuring the patient is not pacemaker-dependent beforehand.
Chronotropic Incompetence and Mortality Risk
The association between CI and increased cardiovascular and all-cause mortality was first reported over 30 years ago by Hinkle et al. [44]. They described a group of men unable to reach expected HR during exercise, who subsequently experienced more cardiac events over 7 years. Initially termed “sustained relative bradycardia,” Rubinstein et al. [45] and Eckberg et al. [46] later linked this phenomenon to autonomic dysfunction. Ellestad et al. [47] confirmed increased cardiac event risk in long-term follow-up and showed that abnormal HR response during exercise carried a greater risk than ischemic ST-segment depression. He proposed the term “chronotropic incompetence” to describe this abnormal HR response.
Numerous subsequent studies have expanded on these findings, demonstrating that attenuated HR response to exercise predicts increased mortality and coronary heart disease risk, independently of age, gender, fitness, cardiovascular risk factors, and ST-segment changes [19, 28, 48, 49]. In a study of over 5,000 asymptomatic women, those with peak exercise HR >1 SD below the predicted mean had markedly increased mortality during long-term follow-up (Figure 5) [19]. Attenuated HR response also predicts myocardial perfusion defects [28]. Combining CI with myocardial perfusion defects during exercise stress testing identifies a particularly high-risk group needing intensified treatment [28]. The prognostic value of impaired HR response persists even after considering adverse effects of coronary artery disease and LV dysfunction [29].
Figure 5. Survival and Peak Heart Rate in Asymptomatic Women
Figure 5: Reduced survival in asymptomatic women with peak HR ≥ 1 SD below average. From Gulati et al, Circulation 2010;122:130-137.
Another study [30] of 3,221 patients undergoing treadmill exercise echocardiography over a median 3.2-year follow-up showed that failure to achieve 85% of maximal predicted HR was associated with increased mortality and cardiac death, even after adjusting for LV function and exercise-induced ischemia. Azarbal et al. [50] found that low % HR reserve was a better predictor than failure to reach 85% APMHR, as the latter identified 2.2 times more individuals at increased cardiac death risk. Attenuated HR response also predicts major adverse cardiac events in individuals with known or suspected cardiovascular disease [51]. In HF patients not on beta-blockers, CI appears to increase mortality risk [52].
In summary, HR profiles during and after exercise are strong predictors of sudden death in asymptomatic and selected clinical populations, including those with coronary artery disease or HF. These findings justify increased screening for inappropriate/inadequate HR responses during exercise testing and recovery for improved risk stratification and prognosis.
Mechanisms of Exercise Intolerance in Heart Failure
Unlike many other heart diseases, heart failure (HF) incidence is increasing, with 500,000 new US cases annually and a 175% rise in HF-related hospital discharges in the past 20 years [53]. A majority of community-dwelling HF patients have preserved LV ejection fraction (HFpEF) [54-56]. A hallmark of both HFrEF and HFpEF is markedly reduced exercise capacity, with peak VO2 15-40% lower than age-matched controls [57]. Studies show HFpEF patients exhibit similar reductions in exercise tolerance (peak VO2), submaximal exercise measures, ventilatory anaerobic threshold, 6-minute walk distance, quality of life, and prognostic markers like VE/VCO2 slope, compared to those with HFrEF [58-61]. These findings have been replicated by Smart et al. and others [62].
According to the Fick equation, adequate VO2 peak increase during exertion relies on both cardiac output increase and arteriovenous oxygen content difference widening [63, 64]. The latter is related to skeletal muscle and vascular abnormalities limiting exercise tolerance in HF [57, 63, 65]. HF patients often achieve less than 50% of the maximal cardiac output of healthy individuals at peak exercise [57]. Reduced cardiac output response in HF correlates significantly with VO2 peak reductions [66]. This reduced response is often attributed to attenuated stroke volume, secondary to systolic or diastolic LV dysfunction. Stroke volume, already diminished at rest in HF, rises only modestly to a peak of 50-65 ml, compared to ≥100 ml in healthy subjects [67]. Consequently, HF patients must rely more on HR increases to augment cardiac output and compensate for inadequate stroke volume during exertion. While maximal HR may be only mildly reduced at peak exercise, HR reserve (HR augmentation above resting levels) is often more substantially blunted in HF due to sympathetically-driven elevated resting HRs [67].
The Contribution of Impaired Heart Rate Response to Exercise Intolerance in Heart Failure
As previously discussed, the Fick equation highlights the dependence of cardiac output increase during exertion on stroke volume and/or HR increases. In both HFrEF and HFpEF, the primary limitation during exertion is often assumed to be the inability to increase stroke volume adequately. However, considering HR responsiveness’s impact on cardiac output and VO2 peak, CI’s role in a population with pronounced exercise intolerance warrants greater attention. A recent study [68] of 102 elderly HFrEF or HFpEF patients demonstrated a significant correlation (r=0.40) between HR reserve (difference between resting and peak HR during bicycle exercise) and VO2 peak (Figure 6). This indicates that HR increase during exercise accounts for a considerable portion (approximately 15%) of VO2 peak differences in these older HF patients. In a patient group with an average VO2 peak of 14 ml/kg/min, abnormal HR accounts for about 2 ml/kg/min (±16%) in VO2 peak, with significant functional and prognostic implications.
Figure 6. Heart Rate Reserve and VO2 peak in Heart Failure Patients
Figure 6: Relationship between heart rate reserve (HRR) and peak exercise oxygen consumption (VO2 peak) in older patients with HFrEF and HFpEF, with and without CI. Significant correlation between HRR and VO2 peak is evident in both groups. From Brubaker, et al, Journal of Cardiopulmonary Rehabilitation 2006; 26:86-89.
Similarly, Witte et al. [37] found VO2 peak significantly lower (by 2.6 ml/kg/min = 14% and 4.6 ml/kg/min = 25%, respectively) in HFrEF patients with CI compared to those without. They also reported a correlation between VO2 peak and delta HR (peak exercise HR – rest HR) of 0.56 and 0.60 for beta-blocked and non-beta-blocked HF patients, further supporting the significant impact of inadequate HR increase during exertion in this population.
Borlaug et al. [69] assessed exercise tolerance parameters in HFpEF patients versus age-, gender-, comorbidity-, and LV hypertrophy-matched controls. At peak exertion, HFpEF patients had significantly reduced VO2 peak (9.0 ± 3.4 vs 14.4 ± 3.4 ml/kg/min) and HR (87 ± 20 vs. 115 ± 22 bpm) compared to controls. Exercise capacity (VO2 peak) correlated directly with cardiac output but was primarily determined by HR and afterload responses during exercise. Changes in end-diastolic volume and stroke volume did not correlate with exercise capacity. HFpEF patients also exhibited slower HR rise, lower peak exercise HR, and impaired HR recovery, indicating autonomic dysfunction (Figure 7) [69].
Figure 7. Heart Rate Profiles in HFpEF Patients During Exercise
Figure 7: Heart rate profiles during and after cycle ergometry in HFpEF patients compared to matched control subjects. Demonstrates delayed and attenuated heart rate response in chronotropically impaired heart failure patients. From Borlaug et al, Circulation 2006; 114:2138-2147.
Prevalence of Chronotropic Incompetence in Heart Failure
Reported CI prevalence in HF varies widely, from 25% to 70%. This variability is likely due to differing diagnostic criteria and patient characteristics (age, disease severity, medications). One of the earliest studies assessing CI prevalence in HF by Clark and Coates [70], using the ≤80% of APHRR criterion, found 66% (14 of 21) of stable, non-beta-blocked HFrEF patients (mean age 54±10 years) had CI. Roche et al. [71] found no significant differences in age, HR, peak oxygen uptake, or LV ejection fraction between HF patients with and without CI. In a slightly older HFrEF group, Witte et al. [37] found 43% (103 of 237) met the <80% APHRR criteria.
A study evaluating older (≥60 years) HFrEF and HFpEF patients and age-matched healthy controls using ≤80% APMHR and the Wilkoff approach [68] found CI uncommon in healthy older adults (7%, 2 of 28), but similarly prevalent in older HFrEF (26%, 12 of 46) and HFpEF (19%, 11 of 56). A more recent unpublished analysis of 207 older HFpEF patients indicated 28% met CI criteria. Phan et al. [42] also observed abnormal HR responses in HFpEF patients compared to age-matched hypertensive and healthy controls. Using <80% of HR reserve, they found CI prevalence of 42% in HFpEF patients, 18% in hypertensive patients, and 9% in healthy controls. Therefore, in addition to central and peripheral derangements in HF, a significant portion (one-third or more, depending on criteria) of both HFrEF and HFpEF populations experience CI, contributing significantly to exercise intolerance.
Mechanisms Underlying Chronotropic Incompetence in Heart Failure
Studies in the 1980s by Bristow et al. [72] and Colucci et al. [73] first linked CI in HF to beta-receptor downregulation and desensitization in the context of elevated circulating catecholamine levels. Bristow et al. [72] found a 50% or greater reduction in beta-adrenergic receptor density in LV myocardium of failing hearts explanted during transplant surgery. Colucci et al. demonstrated that norepinephrine infusion resulted in a reduced HR response in HF patients compared to healthy subjects [73]. During maximal isoproterenol stimulation, Bristow et al. observed a 45% reduction in adenylate cyclase elaboration and up to 73% reduction in muscle contraction compared to normal. These findings suggest that in HF, decreased beta-receptor density leads to reduced beta-adrenergic pathway sensitivity and decreased beta-agonist-stimulated muscle contractility [72]. Samejima et al. [74] showed that the ratio of HR change to log norepinephrine change (delta HR/delta log NE), an index of sinoatrial node sympathetic responsiveness, decreased progressively with HF severity. Furthermore, the delta HR/log delta NE ratio during exercise correlated significantly with anaerobic threshold, VO2 peak, and VE/VCO2 slope [74]. An electrophysiology study [75] of symptomatic HFrEF patients and age-matched controls undergoing radiofrequency ablation for AV tachycardia or AV nodal tachycardia revealed sinus node remodeling in HF patients without prior atrial arrhythmias. This remodeling included (1) anatomical and structural changes along the crista terminalis, (2) prolonged sinus node recovery and sinoatrial conduction, and (3) caudal localization of the sinus node complex with circuitous impulse propagation. This reduction in sinus node reserve appears to contribute to bradycardia and CI commonly seen in HF [75].
Management Strategies for Chronotropic Incompetence in Heart Failure
Exercise Training: Endurance exercise training in healthy individuals yields favorable chronotropic function changes, including decreased resting and submaximal exercise HR, and faster post-exercise HR decline. These adaptations are largely due to altered sympathetic-parasympathetic balance. Exercise training generally improves exercise tolerance in HFrEF patients via central and peripheral mechanisms. Specific effects on autonomic dysfunction and neurohormonal activation in chronic HF include increased baroreflex sensitivity and HR variability, and reduced sympathetic outflow, plasma catecholamine, angiotensin II, vasopressin, and brain natriuretic peptide levels at rest [76, 77]. Exercise training appears to modify abnormal afferent stimuli from the failing heart that increase sympathetic outflow, leading to autonomic derangement and neurohumoral activation [76]. Hasking et al. [78] found that plasma norepinephrine concentrations were elevated in asymptomatic LV dysfunction patients and increased further with progression to overt HF; in later HF stages, total body spillover was double that of controls, and norepinephrine clearance reduced by a third. While beneficial, the precise mechanisms of exercise training’s modification of neurohormonal activation and autonomic derangement in HF remain unclear.
Several exercise training studies [79-81] have shown that peak exercise HR increases by 5-7%, contributing to increased cardiac output and VO2 peak in HF patients. A meta-analysis of 35 randomized exercise training studies in HF patients [82] indicated an average peak HR increase of 4 bpm, or 2.5% of pre-training levels. Keteyian et al. [81] demonstrated a 7% (approximately 9 bpm) peak exercise HR increase after 24 weeks of endurance training, with no change in controls. Training-induced peak HR increase accounted for 50% of the VO2 peak increase (+2 ml/kg/min = 14%) in the exercise group. While beta-adrenergic receptor sensitivity changes may explain these findings, other mechanisms contributing to improved chronotropic response in HF cannot be excluded. Further research is needed on exercise training’s impact on chronotropic response in HFrEF and HFpEF.
Rate Adaptive Pacing: A linear relationship exists between HR and VO2 during exercise in various populations, including HF [83], where a 2-6 bpm HR increase is associated with a 1 ml/kg/min VO2 increase. Rate-adaptive pacing has been shown to enhance functional capacity in patients with inadequate chronotropic response [84] and CI [32, 85]. Despite its potential to improve HR, cardiac output, and VO2 during exertion in CI-related HF, rate-adaptive pacing has received limited attention [86, 87]. Some clinicians may find it counterintuitive to use pacemakers for HF patients, especially without bradycardia or heart block.
Tse et al. [88] assessed rate-adaptive pacing combined with cardiac resynchronization therapy on exercise performance in HFrEF patients with CI. Twenty HFrEF-CI patients (CI defined as <80% APMHR) underwent treadmill testing with VO2 measurement. Cardiac resynchronization devices were programmed to: 1) DDD mode with fixed AVI (DDD-off); 2) DDD mode with AVI algorithm on (DDD-ON); and 3) DDDR mode. None achieved >85% APMR, and 55% failed to reach >70% APMHR (severe CI). Overall, rate-adaptive pacing during cardiac resynchronization increased peak exercise HR and time, but not VO2 peak. However, in patients with more severe CI (<70% APMHR), rate-adaptive pacing significantly increased VO2 peak (2.04 ± 0.46 vs. 1.74 ± 0.41 ml/kg/min, p<0.05). In most (82%) of these severely CI patients, improved chronotropic response with rate-adaptive pacing was associated with ~20% VO2 peak increase.
It is important to note that patients with less severe CI (70-85% APMHR) experienced little to no benefit, and one-third even had reduced exercise capacity with rate-adaptive pacing [88]. While rate-adaptive pacing shows promise in carefully selected HFrEF patients, progress is limited by lack of standardized CI definitions and selection criteria. It remains unclear if CI is causal or just a marker of advanced disease, and whether pacing improves functional status in HFrEF. Further investigation is crucial.
Even less is known about pacing in HFpEF. The ongoing RESET trial is evaluating rate-adaptive pacing in HFpEF patients with overt CI [39]. Rationale stems from observations that ~30% of this population has CI, significantly contributing to objectively measured exercise intolerance [42, 68, 69]. This randomized controlled trial may determine rate-responsive pacing’s effectiveness in improving exercise function in HFpEF.
Conclusions and Suggested Approach to Assessment and Management
Chronotropic incompetence is a common and significant cause of exercise intolerance and an independent predictor of major adverse cardiovascular events and mortality. It affects up to one-third of HF patients, contributing to exertional symptoms and reduced quality of life. While mechanisms are not fully understood, reduced beta-receptor density/sensitivity secondary to increased sympathetic drive likely plays a role [89].
CI diagnosis should account for age, physical condition, and medications, but can be objectively achieved with exercise testing and standardized definitions. A three-step assessment approach is suggested: 1) Perform a progressive, exhaustive, symptom-limited exercise test, ideally with expired gas analysis for RER and peak VO2. 2) Use a relevant peak heart rate formula (Tanaka for healthy individuals [21], Brawner for CVD or beta-blocker users [22]). 3) If the patient fails to achieve 80% of APMHR despite maximal effort (RPE, symptoms, RER), calculate the Wilkoff Chronotropic Index. If CI is confirmed, investigate reversible causes.
In HF, beta-blockers may have less detrimental effect on exercise capacity than previously thought, possibly even paradoxically improving performance. Beta-blockers and negative inotropes do not appear to significantly impact HR response in HF, making separate CI criteria for these patients potentially unnecessary. Beta-blockers may not substantially increase CI prevalence in HF. The potential of newer beta-blockers to reduce CI prevalence in HF is unclear.
Exercise training and rate-adaptive pacing can improve chronotropic responses and exercise capacity in HF, but more research is needed to fully evaluate their impact on clinical outcomes.
CI is a common, easily diagnosed, and potentially treatable cause of exercise intolerance, warranting increased clinical attention in patients with exertional symptoms.
Acknowledgement
We gratefully acknowledge Rickie Henderson, MD, for critical review of the manuscript and Belinda Youngdahl for administrative assistance.
Both authors participated in drafting of the manuscript, reviewed and edited the manuscript for critical intellectual content, and approved the final version for submission. The sponsor had no role in design and conduct of the study; collection, management, analysis, and interpretation of the data; or preparation, review, or approval of the manuscript.
Funding Sources
Supported in part by: National Institutes of Health grants R37AG18915 and P30AG21332.
Footnotes
The authors wish to declare the following potential conflicts of interests or financial disclosures
Dr. Brubaker: Boston Scientific (> $10K)
Dr. Kitzman: Synvista (> $10K), Bristol-Meyers-Squibb (> $10K), Novartis (> $10K), Boston Scientific (> $10K), Relypsa (> $10K), Forest Laboratories (
This is a PDF file of an unedited manuscript that has been accepted for publication. As a service to our customers we are providing this early version of the manuscript. The manuscript will undergo copyediting, typesetting, and review of the resulting proof before it is published in its final citable form. Please note that during the production process errors may be discovered which could affect the content, and all legal disclaimers that apply to the journal pertain.
References
[1] Myers J, Prakash M, Froelicher V, Do D, Partington S, Atwood JE. Exercise capacity and mortality among men referred for exercise testing. N Engl J Med. 2002;346:793-801. [ PubMed ]
[2] Rowell LB. Human cardiovascular control. New York: Oxford University Press; 1993.
[3] Lakatta EG. Age-associated cardiovascular changes in health: impact on cardiovascular disease in older persons. Heart Fail Rev. 2002;7:29-49. [ PubMed ]
[4] Wilkoff BL, Miller RE. Chronotropic incompetence: mechanisms, manifestations, and implications. Pacing Clin Electrophysiol. 1994;17:1565-75. [ PubMed ]
[5] Jouven X, Empana JP, Schwartz PJ, Desnos M, Courbon D, Ducimetiere P. Heart-rate profile during exercise as a predictor of sudden death. N Engl J Med. 2005;352:1951-8. [ PubMed ]
[6] Diaz A, Bourassa MG, Guertin MC, Tardif JC. Long-term prognostic value of resting heart rate in patients with suspected or proven coronary artery disease. Eur Heart J. 2005;26:967-74. [ PubMed ]
[7] Bohm M, Swedberg K, Reil JC, Komajda M, Borer JS, Ford I, et al. Heart rate as a risk factor in chronic heart failure (COMMIT HF). Eur Heart J. 2008;29:2137-43. [ PubMed ]
[8] Lauer MS. Heart rate recovery: a novel, powerful, yet underutilized clinical tool. Cleve Clin J Med. 2009;76 Suppl 2:S18-22. [ PubMed ]
[9] Cole CR, Blackstone EH, Pashkow FJ, Snader CE, Lauer MS. Heart-rate recovery after submaximal exercise testing as a predictor of mortality in a cardiovascularly healthy cohort. Ann Intern Med. 2000;132:572-5. [ PubMed ]
[10] Imai K, Sato H, Hori M, Kusuoka H, Ozaki H, Yokoyama H, et al. Vagally mediated heart rate recovery after exercise is accelerated in athletes but blunted in patients with chronic heart failure. J Am Coll Cardiol. 1994;24:1529-35. [ PubMed ]
[11] Lauer MS, Okin PM, Larson MG, Evans JC, Levy D. Impaired heart rate recovery after exercise stress testing and prediction of sudden cardiac death. Circulation. 1999;100:1352-8. [ PubMed ]
[12] Vivekananthan DP, Blackstone EH, Pothier CE, Lauer MS. Heart rate recovery after exercise: relations to heart rate variability and time-domain measures of autonomic function. Am Heart J. 2003;146:827-32. [ PubMed ]
[13] Kligfield P, Lauer MS. Exercise electrocardiogram testing: still valuable after all these years. Cleve Clin J Med. 2009;76 Suppl 2:S3-11. [ PubMed ]
[14] Laukkanen JA, Kurl S, Salonen R, Rauramaa R, Salonen JT. Blunted heart rate increase during exercise and exaggerated blood pressure response as predictors of cardiovascular mortality in middle-aged men. J Hypertens. 2006;24:313-20. [ PubMed ]
[15] Jose AD, Collison D. The effect of aging and beta blockade on intrinsic heart rate and maximal heart rate. Cardiovasc Res. 1970;4:160-7. [ PubMed ]
[16] Hossack KF, Bruce RA. Maximal cardiac function in sedentary men and women: comparison of age-related changes. J Appl Physiol. 1982;53:799-804. [ PubMed ]
[17] Rodeheffer RJ, Gerstenblith G, Becker LC, Fleg JL, Weisfeldt ML, Lakatta EG. Exercise cardiac output is maintained with advancing age in healthy human subjects: cardiac dilatation and increased stroke volume compensate for diminished heart rate. Circulation. 1984;69:203-13. [ PubMed ]
[18] Higginbotham MB, Morris BL, Williams RS, Coleman RE, Cobb FR. Regulation of stroke volume during upright exercise in normal subjects. Circ Res. 1986;58:281-91. [ PubMed ]
[19] Gulati M, Shaw LJ, Thisted RA, Black HR, Merz CN, Arnsdorf MF. Heart rate response to exercise stress testing in asymptomatic women: the St James Women Take Heart Project. Circulation. 2010;122:130-7. [ PubMed ]
[20] Froelicher VF, Myers J, Follansbee WP, Labovitz AJ. Exercise and the heart. 4th ed. Philadelphia: Saunders; 2000.
[21] Tanaka H, Monahan KD, Seals DR. Age-predicted maximal heart rate revisited. J Am Coll Cardiol. 2001;37:153-6. [ PubMed ]
[22] Brawner CA, Marwick TH,摘自: Myers J, Ashley EA, Vogel-Spar